Epigenetics: how your parents’ experience can influence your DNA
Genetic inheritance is more than just a DNA sequence. Certain molecular mechanisms remember your experience on the level above the genes. Let’s have a closer look at epigenetics.
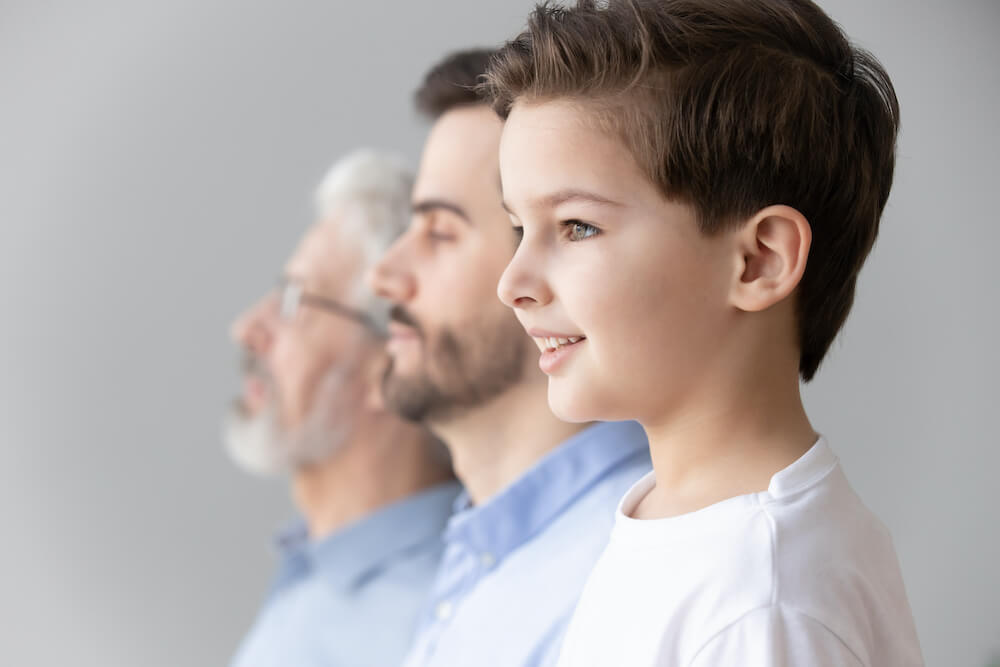
No one has perfect genetics. Anyone who does is either a superhuman or a liar!
Our DNA sequence is created by combining one from the mother and one from the father. And every time you wish you were a little bit taller, a little bit shorter, or a little bit less addicted to tomato juice—you blame your parents’ genes, don’t you? Don’t worry, we all do that!
But there is more to genetic inheritance than just the genes—that is, the sequence of nucleotides, long molecules that make up the DNA. There is something more that also can be passed down through generations. The mechanisms that influence the genes on the molecular level are called epigenetic, where “epi-” means “on the outside of.” As you can guess from the name, epigenetics affect genes outside of the DNA sequence, the traditional mechanism of inheritance.
Certain molecules (we call them methyl groups) can turn genes “on” and “off.” The pattern of these molecules’ behavior can also be inherited.
We’ll explore fascinating examples of how these DNA-regulating molecules work:
- the fear that Holocaust survivors passed on to their offsprings;
- the influence of the father’s lifestyle on a future child;
- the identical twins with the same DNA who grow up different;
- the knowledge of when to ripe that tomatoes pass on across generations.
All of these are evidence ofDNA methylation—the process of adding methyl groups to certain sections of the DNA.
We have begun to discover epigenetic mechanisms in the 1990s, and today there is still much unexplored and unexplained in this field. Methylation is the mechanism we know the most about.
Why and how do we turn genes on and off?
But before we get to the place outside of the genes, let’s look at the very beginning—the conception. We’ll skip the uninteresting details up to the point when the father’s DNA enters the mother’s ovule to create a zygote.
This cell is ready to divide, and multiply, and transform into every kind of cell that is there in the human body.
From this zygote, many new cells are created and transformed into different types.
Pretty soon, the zygote develops into an embryo, which has many different types of tissue. These tissues further develop into organs.
But how does the zygote make different types of cells from many identical ones? The instructions on the transformation are encoded within the genes—which it got from the parents. In order to make tissue, a particular set of genes has to be activated in the particular area of the embryo.
For example, a set of genes responsible for forming cardiomyocytes (heart muscle cells) should be activated in the area where the heart should be developed—and not anywhere else.
So, is there anything that controls the activation of the genes? Indeed there is! Specific molecules can turn the genes “on” and “off” in the right place at the right time.
Some scientists have been working with identical mice embryos. They managed to activate the “leg development” genes in the place where the mouse embryo should have developed its tail.
Do you think it worked? Well, here’s a photo of a mouse with an additional leg instead of a tail.
So, how did that happen exactly?
There are chemicals called methyl groups. When bound to the nucleotides, they can affect gene activity.
If you add a methyl group to the start of a gene sequence, this gene can’t be “read.” This is one of the most common ways to control gene activation.
Thus, by introducing methyl groups to certain places of a mouse’s DNA, the scientists made the cells develop into “leg cells” rather than “tail cells.” And that’s how the mouse got a leg instead of a tail.
The process of adding methyl groups to the DNA is calledDNA methylation. It’s not something only scientists can do—our bodies do it all the time.
Methylation doesn’t change the code of the DNA sequence itself, only the way it’s activated. That’s why we consider it anepigenetic mechanism. There are other epigenetic mechanisms. Genes can be regulated by modifying the histones—proteins that hold the DNA structure. The way DNA is wrapped around the histone in the nucleus (DNA packaging) can also affect its activation. RNA-molecules (which are similar in structure to the DNA) can signal additional information about how to handle the genes.
So, we have learned that in order to have our bodies properly developed, it is not enough just to encode genetic information—the genes need to “know” when to activate.
One of the ways to do this is to bind a methyl group to a part of the DNA molecule, silencing a certain gene. This is called DNA methylation, and it is, perhaps, the most important and explored mechanism of all epigenetics. It is related to many crucial processes in our bodies’ growth, development, and functioning.
Next up, we’ll explore methylation in more detail.
Embryo development and epigenetics
So, many fascinating things can happen thanks to methylation—not only the mouse tail-leg.
There are four types of nucleotides—“building blocks” for the DNA. Methyl groups can bind to the cytosine-guanine connection, known as “CG sites.”
Humans have up to 80% of CG sites in their DNA methylated—though the number is different at different stages of our lives. Other species can also have different levels of DNA methylation.
Let’s go back to the embryo we’ve left in the previous section. One of the important steps a fertilized zygote goes through is demethylating its DNA—that is, erasing almost every methyl group from the genes.
Remember that the zygote starts as only a single cell? Demethylation allows it to erase the memory of the parental DNA and transform into any cell. It’s interesting that the father’s and the mother’s DNA are demethylated at different speeds!
However, there are several points in the development of the zygote when parental methylation patterns are copied and established anew. To create a new organism, parental DNA should reset these patterns first, but then the zygote has to set them again.
This process is crucial forcell differentiation, which means developing different kinds of cells from the initial one. Various methylation patterns in different cells activate the genes for particular tissue types.
And now something exciting happens!
The zygote receives two DNA sequences—the mother’s and the father’s. The sequences all have similar genes, just encoded differently. This means each one of us has two different copies of every gene in the DNA.
Usually, our bodies use both variants of a gene—except for some genes that are forever methylated and can’t be activated at all. We call them imprinted genes, and to date, there have been 228 such genes found in humans.
Thus, some genes are activated (as scientists say, expressed) only from the father’s DNA or the mother’s one.
For instance, a gene responsible for the growth hormone activity, which is critical during pregnancy, is expressed only from the father’s DNA.
When the embryo grows into an adult human, its germ cells—sperm or oocytes—become reimprinted. Male spermatozoids and female oocytes have different imprinting patterns.
This way, the imprints are passed on down to the grandchildren and further. We can use these imprints as evidence of some events in the family history.
But the imprinting patterns are very conservative, and changes in them are rare. Other methylation patterns are much more dependent on the environment and life experience.